Star Collisions Forge the Universe’s Heaviest Elements
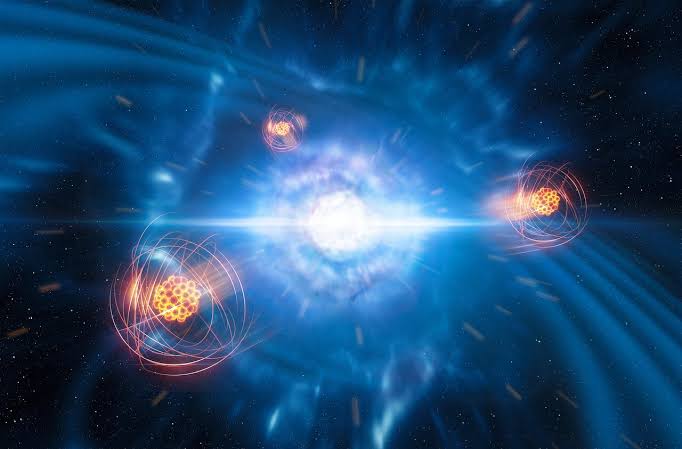
Bits of the stars are all around us, and in us, too. About half of the abundance of elements heavier than iron originates in some of the most violent explosions in the cosmos.
As the universe churns and new stars and planets form out of old gas and dust, these elements eventually make their way to Earth and other worlds.
After 3.7 billion years of evolution on our planet, humans and many other species have come to rely on them in our bodies and our lives. Iodine, for instance, is a component of hormones we need to control our brain development and regulate our metabolism.
Ocean microplankton called Acantharea use the element strontium to create intricate mineral skeletons. Gallium is critical for the chips in our smartphones and our laptop screens. And the mirrors of the JWST are gilded with gold, an element useful for its unreactive nature and ability to reflect infrared light (not to mention its popularity in jewelry).
Scientists have long had a basic idea of how these elements come to be, but for many years the details were hazy and fiercely debated. That changed recently when astronomers observed, for the first time, heavy-element synthesis in action. The process, the evidence suggests, went something like this.
Eons ago a star more than 10 times as massive as our sun died in a spectacular explosion, giving birth to one of the strangest objects in the universe: a neutron star. This newborn star was a remnant of the stellar core compressed to extreme densities where matter can take forms we do not understand.
The neutron star might have cooled forever in the depths of space, and that would have been the end of its story. But most massive stars live in binary systems with a twin, and the same fate that befell our first star eventually came for its partner, leaving two neutron stars circling each other. In a dance that went on for millennia, the stars spiraled in, slowly at first and then rapidly. As they drew closer together, tidal forces began to rip them apart, flinging neutron-rich matter into space at velocities approaching one-third the speed of light. At last the stars merged, sending ripples through spacetime and setting off cosmic fireworks across the entire electromagnetic spectrum.
How Star Collisions Forge the Universe’s Heaviest Elements?
At the time of the crash, our own pale blue planet, in a quiet part of the Milky Way about 130 million light-years away, was home to the dinosaurs. The ripples in spacetime, called gravitational waves, began making their way across the cosmos, and in the time it took them to cover the vast distance to Earth, life on the planet changed beyond recognition.
New species evolved and went extinct, civilizations rose and fell, and curious humans began looking up at the sky, developing instruments that could do incredible things such as measure minute distortions in spacetime. Eventually the gravitational waves (traveling at light speed) and the light from the merger reached Earth together.
Astrophysicists recognized a distinctive glow that showed the presence of new elements. Humanity had just witnessed heavy-element production.
The discovery has answered several long-standing questions in astrophysics while also raising entirely new questions. But many scientists are energized. Their newfound ability to detect gravitational waves, as well as light from the same cosmic source, promises to help them understand astrophysical explosions and the synthesis of elements in a way that was previously impossible.
The quest to understand heavy-element formation is part of a larger scientific effort to answer a fundamental question: Where did everything come from? The cosmic history of the elements of the periodic table extends from a few minutes after the big bang to the present.
The synthesis of the first elements—hydrogen, helium and lithium—occurred roughly three minutes after the birth of the universe. From these ingredients, the first stars formed, shining bright and fusing new elements in their cores during both their lives and their explosive deaths. The next generation of stars was born from the debris of these blasts, enriched with the elements formed by the first stars.
This process continues today and accounts for all the elements from helium on the light end, with two protons per atom, all the way up to iron, which has 26 protons in its atomic nucleus.
The heaviest elements, such as tennessine with 117 protons, aren’t created by nature at all. But physicists can force them into being inside particle accelerators, where they typically last for mere thousandths of a second before decaying.
We all are star dust
Several decades ago scientists theorized that about half of the elements heavier than iron are produced through a process called rapid neutron capture, or the r-process. The rest are thought to originate through slow neutron capture, or the s-process—a relatively well-understood sequence of reactions that occurs in long-lived, low-mass stars.
Both the r-process and the s-process involve adding one or more neutrons to an atomic nucleus. Adding neutrons, however, does not produce a new element, because elements are defined by the number of protons in their nucleus. What we do get is a heavier isotope of the same element—a nucleus containing the same number of protons but a different number of neutrons.
This heavy isotope is often unstable and radioactive. Through what’s called beta-minus decay, a neutron will transform into a proton, spitting out an electron and another subatomic particle called a neutrino in the process. In this way, the number of protons in an atom’s nucleus increases, and a new element is born.
The key difference between the s-process and the r-process is speed. In the s-process, atoms capture neutrons slowly, and there is plenty of time for the newly added neutron to decay into a proton, creating the next stable element in the periodic table—with just one proton more—before another neutron comes along to be captured.
This happens over thousands of years because there are only small numbers of extra neutrons lying around in the stars that host the s-process, so atoms are able to capture new neutrons only occasionally.
The r-process, in contrast, can produce the entire range of heavy elements in one spectacular flash of creation that barely lasts a second. In this scenario, neutrons are plentiful and slam into nuclei one after another before they have time to decay. A nucleus can rapidly balloon into a highly unstable isotope, going all the way up to what’s called the neutron drip line—the absolute limit of the neutron-to-proton ratio allowed by nature inside a nucleus.
The extremely heavy nucleus will then convert many of its neutrons to protons via beta decays or even break into smaller nuclei, ultimately producing a range of stable heavy elements. Many details about how this plays out are unclear.
After a nucleus absorbs extra neutrons, for instance, but before it becomes stable, exotic nuclei arise that scientists don’t understand. These in-between nuclei have properties that push the bounds of physics, and measuring them in a laboratory is difficult and sometimes even impossible.